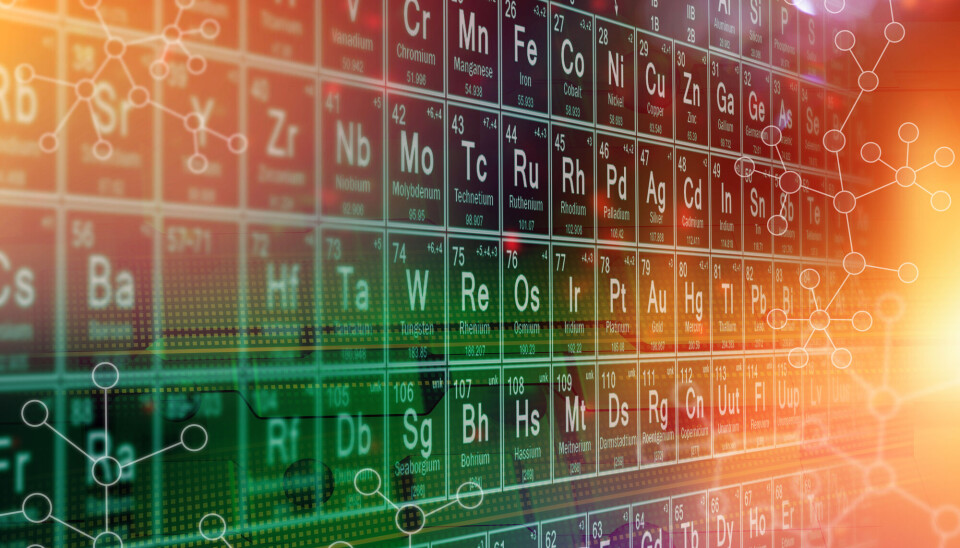
Centenary for the discovery of element 72 – a Nordic contribution and the utility of hafnium
(Sub- and superscript numbers are not visible in the main text (only in the figure). A PDF-file with sub- and superscripts can be downloaded on this link)
On January 20, 1923 Coster and Hevesy reported their discovery of the element hafnium (Hf) in Nature. Because the element was identified in the mineral zircon at the Niels Bohr Institute, they named it after Copenhagen's latin name, Hafnia.
The famous Norwegian geochemist, Victor M. Goldschmidt, conducted a parallel search for element 72 in Oslo. With his co-investigator, L. Thomassen, he also recorded it in zircon samples, 29 days later than the Copenhagen group.
Background
When Dimitri Mendeleev systemised the chemical properties of the elements via a «periodic table» in 1869, there were several undiscovered elements. In 1922, hafnium (72Hf) and rhenium (75Re) were the only two remaining stable elements not yet identified. The periodic table below represents the state of the art about 150 years after Medeleev's pioneering ground work.
In 1912, Max van Laue found that X-rays are diffracted by crystals. Henry Moseley (1887-1915), working in Rutherford's group at the University of Manchester, immediately utilised this discovery to investigate the unique characteristics of the fluorescent X-ray emmision of each of the chemical elements when bombarding them with electrons.
Moseley published his X-ray spectroscopy results in two important articles in the Philosophical Magazine in December 1913 and May 1914. The latter article tabulated the elements from aluminum (13Al) to gold (79Au), and emphasized that their X-ray spectroscopic features were uniquely linked to the atomic number of each element. Moseley's elemental sequence had three gaps for elements 43, 61 and 75.
Already in 1911, the French geochemist Georges Urbain (1872-1938) thought that he had discovered element 72, which he named celtium. Urbain visited Moseley in June 1914 and brought with him some of his celtium separates.
Immediately, Moseley found that the X-ray spectra demonstrated that those separates contained mixtures of the two heaviest lanthanides (rare Earth elements), ytterbium (70Yb) and lutetium (71Lu). Soon after that, the First World War broke out, and Moseley enlisted in the British army.
Rutherford's urgent request that Moseley ought to be transferred from active combat to the National Physics Laboratory came too late. He was sent to Gallipoli and killed by a Turkish sniper on August 10, 1915.
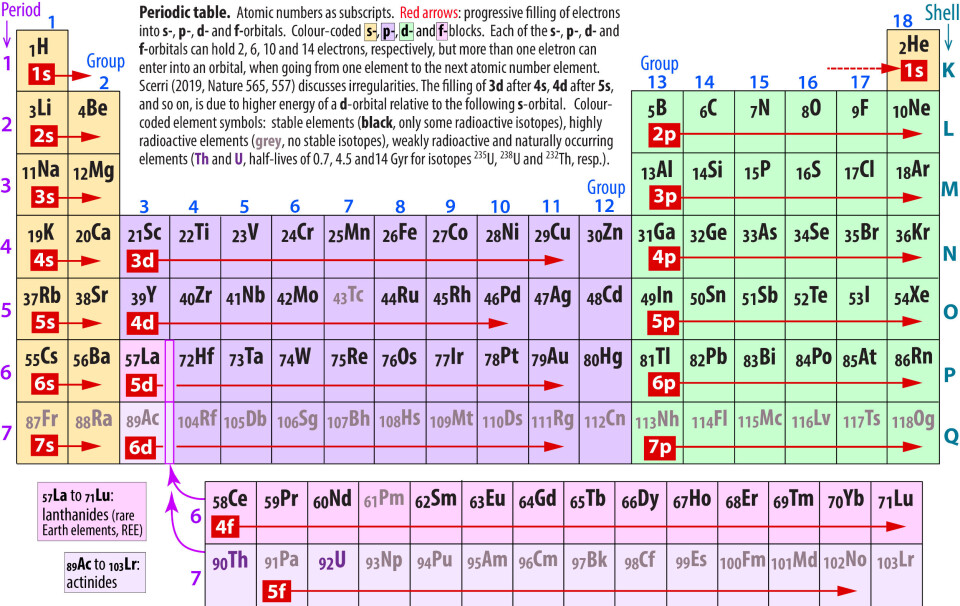
High-resolution PDF-file with model
The Nordic search
The search for element 72 intensified in 1922, mainly in the research groups of Niels Bohr and Victor M. Goldschmidt in Copenhagen and Oslo, respectively. Based on the periodic table position of element 72 in group 4 between the trivalent lanthanides (57La to 71Lu) and the pentavalent tantalum (73Ta), and directly beneath the quadrivalent zirconium (40Zr), both of the groups focussed on Norwegian samples of the mineral zircon, with composition close to ZrSiO4.
The Bohr group used Norwegian zircon samples from the collections in the Geological Museum in Copenhagen, while Goldschmidt, based at the Geological Institute and later at the Mineralogical-Geological Museum, University of Oslo, used his own zircon samples.
Both groups had developed X-ray spectroscopic methods and succeeded in their searches, 29 days apart. Coster and Hevesy (the Bohr group) submitted an article to Nature on January 2, 1923 (published January 20), whereas Goldschmidt and Thomassen submitted their results on January 31, 1923 to the Norwegian Journal of Geology (Norsk geologisk tidsskrift).
Before their X-ray spectroscopic analysis, Coster and Hevesy (1923) concentrated zirconium and hafnium by boiling the zircon mineral samples in acid to remove most of the soluble components (Mason 1992). A complete separation of hafnium from zirconium is difficult, and because Hf occurs mainly as a minor element in Zr-rich minerals, Goldschmidt classified it as a «camouflaged» element (Mason 1992).
The very nice fit of Hf4+ into the crystal structure positions hosted mainly by Zr4+ is related to the socalled «lanthanide contraction»: The ionic sizes of the trivalent lanthanides preceding Hf in period 6 decrease with increasing atomic number from 57La to 71Lu due to poor nuclear shielding by the 4f-orbital electrons.
The outer electrons in the very incomplete O- and P-shells are therefore efficiently pulled inwards by the increasingly positive nuclear charges of the increasingly heavier lanthanides up to 71Lu.
The «lanthanide contraction» also results in a slightly smaller quadrivalent ion radius of 83 picometer for 72Hf in 8-coordinated positions, compared to 84 picometer for the much lighter 40Zr (Shannon and Prewitt, 1970; Shannon, 1976). The corresponding atomic radii for Hf and Zr are 156.4 and 159.0 picometer, respectively.
Whereas natural zircon crystals commonly have about 2.4 mol per cent of the HfSiO4 endmember component (the hafnon component, e.g. Speer and Cooper, 1982), some varieties contain more that 30 mol per cent of this component. Goldschmidt sent a zircon sample of the alvite variety with about 15 mol per cent hafnon to Hevesy in Copenhagen.
Generally, the mineral name hafnon could be used for solid solutions with more than 50 mol per cent of the HfSiO4 component, e.g. a variety with more than 90 mol per cent hafnon component from tantalum-rich pegmatites in Mozambique (Correira Neves and Lopes Nunes). The close Zr-Hf association is very useful in geochemical investigation of the ubiquitous and resistant trace mineral zircon (see the section on «Isotope cosmochemistry and geochemistry», below).
Aftermath
Hafnium turned out to be the second last of all the stable elements to be discovered. Element 75, rhenium, named after the river Rhine, was discovered only 2 years later by Noddack and Tacke (1925). Long before that, in 1908, Masatake Ogawa, in a search for element 43, discovered element 75 rhenium, which is located just below element 43 in group 7 of the periodic table (figure above).
Ogawa named it nipponium, assuming that it was element 43. This interesting story is recorded by Hisamatsu et al. (2022). Element 43, however, which is now named technetium (Tc, for the Greek term for artificial), has only short-lived radioactive isotopes with maximum half-lives of about 4 million years. Therefore, Tc is almost absent from natural materials.
Goldschmidt was greatly disappointed after losing the «close race» to the element 72 discovery (Mason 1992). In the following years he devoted much time and effort to search for the rare Earth element 61 in the large collection of lanthanide-rich minerals in the Mineralogical-Geological Museum in Oslo.
After about ten years he realized that element 61 probably has no stable isotope and cannot be found in nature. His inference was confirmed in 1945 by the detection of element 61 (then named promethium, Pm) in fission products of uranium, irradiated in a graphite reactor at Oak Ridge National Laboratory (e.g. Marshall and Marshall, 2016).
Technological applications of hafnium
With its high melting point, corrosion resistance, neutron-capture ability and excellent mechanical properties, hafnium is used in control rods in nuclear reactors, and especially under the harsh conditions of pressurized water reactors.
In order to increase corrosion resistance, as well as the melting point, Hf is used in metal alloys, primarily with Fe, Ti, Nb and Ta. Such alloys are used in e.g. in the nozzles of rocket thrusters. Hafnium-based materials are also used in microprocessors. Its heat resistance and affinity for oxygen and nitrogen, make Hf a good scavenger for O and N in incandescent light bulbs. Because of its ability to loose electrons into air, Hf is also suitable as electrodes in plasma-cutting devices.
The challenging and expensive process of Hf-separation from the far more abundant Zr «host» has, however, limited the technical use of Hf.
Isotope cosmochemistry and geochemistry
Hafnium plays important roles in one short-lived and one longlived radioactive decay scheme. The short-lived decay of 182Hf to 182W, which has a 8.9 My (million years) half-life, operated mostly in the first 40-50 My of our solar system, and in particular in the first 5-10 My.
The initial melting and segregation of Fe-dominated metallic cores in planetesimals occurred mostly in the first 3-5 My, prior to the growth of planetary embryos and planets in the following 200 My. In the very beginning, dense liquid metal alloys dominated by Fe and Ni separated efficiently from the overlying magma ocean to form planetary cores.
Hafnium is «lithophile» ("lithos" means rock or rocky in Greek) and remains in the silicate magma oceans, which gradually crystallises to the rocky mantles and crusts, whereas tungsten (W) partitions into the segregating metal.
The results of this partitioning, if it happens well before 182Hf is extinct, is that the mantles grow high 182W/184W ratios, whereas the cores have low 182W/184W ratios (Lee and Halliday 1996; Halliday 2000, Kruijer et al 2012, 2015, 2021; Kruijer and Kleine 2017; Touboul et al. 2015). In these ratios, 184W is a normalising, stable and the most abundant W-isotope.
An important realization from the Hf-W isotope systematics is that much of the core-mantle segregation in the inner terrestrial planets, including the asteroid and planetesimal, Vesta, occurred very early and mostly at the planetesimal stage, while the radioactive decay of 26Al to 26Mg (half-life, th of only 0.7 My) was the main heat source.
Currently, we have analysed samples and meteorites from Earth, Moon, Mars and Vesta, in addition to numerous other meteorites. Later, when planetary growth continued by numerous collisions between planetesimals and planetary embryos, the already-formed cores in the colliding bodies merged with each other without very extensive re-equilibration with the silicate magma oceans. This happened because the molten metal alloys, with almost twice as high density as the silicate magma, sank rapidly inwards to the main core as one or several large blobs.
Based on a slightly lower 182W/184W ratio in some ocean island basalts (from e.g. Iceland, Azores, Hawaii, Galapagos and Samoa) compared to the uniform ratio in most of the mantle and crust, we recognise a weak signal of core-contamination in the root-zones of most of the Earth's major hot upwellings.
Therefore, these columnar mantle plumes, which undergo partial melting in the uppermost mantle to produce ocean island volcanism, seem to originate at the core-mantle boundary (Mundl et al. 2017; Mundl-Petermeier et al. 2020).
The long-lived decay involving Hf is that of 176Lu to 176Hf (th of 36000 My = 36 Gy). With the age of 4.567 Gy for the Solar system and about 4.36 Gy for the Moon and the fully grown Earth (e.g, Borg and Carlson 2023), the Lu to Hf decay system is still within the first 12-13 per cent of its first half life.
This system is a useful tracer primarily for the crust and mantle (Amelin et al. 1999; Bauer et al. 2020). During partial melting in the mantle, the radioactive parental element, Lu, is held in the residue, especially in garnet, if present, to a greater extent than the radiogenic daughter element, Hf, which partitions strongly into the melt.
Because planetary crusts are formed from mantle melts, their 176Hf/177Hf ratios grow slowly with time, whereas the 176Hf/177Hf ratios of melt-depleted mantles grow faster (Amelin et al. 1999). Here we use 177Hf as the stable and normalizing Hf-isotope.
The time-integrated tracer capability of the Lu-Hf decay system is very useful in combination with 235U to 207Pb (relatively short th of 0.7 Gy) and 238U to 206Pb (th of 4.5 Gy) decay schemes. When cystallising, zircon has U- and Th-concentrations of 10-1000 ppm (parts per million in mass) and Hf-concentrations up to several mass per cent, but very low contents of Pb and Lu.
Therefore, individual zircon grains (or even smaller parts of such grains) in continental crust, may yield internally consistent and accurate 235U-207Pb and 238U-206Pb ages for the crust and potentially an estimate of the 176Hf/177Hf ratio in the source mantle at the time when the mantle-derived melts were extracted to form that specific crustal segment (Amelin et al. 1999; Bauer et al. 2020).
The latter interpretation depends on whether one can reasonably assume that the zircon crystals formed near the time of mantle-derivation of that crust. In many cases it is also possible to unravel some of the crustal evolution complexities even if the zircon minerals are formed after remelting of previously formed crust.
Implications for Earth and planetary evolution
During the last two decades, in particular, the isotope geochemistry of hafnium with its daughter and parent isotopes, recorded in Solar System samples, has contributed materially to our insights into Earth and planetary evolution, structure and dynamics.
In a broad interdisciplinary context, such geochemical results are now integrated with results from experimental and first principles theoretical mineral physics and geochemistry, seismological, gravitational, geodetic and magnetic data from Earth, Moon and Mars, as well as variable gamma-ray spectrometry, gravity, shape and moment of inertia observations from pass-by and orbiting spacecrafts at e.g. Mercury, Vesta, Ceres, several other asteroids, and moons, including those of the Jupiter and Saturn, as well as the Pluto-Charon system.
Such data are, and will increasingly be, integrated into «geodynamic» models. Back in 1923, Coster, Hevesy, Goldschmidt and Thomassen could not have foreseen the range of the geochemical and cosmochemical impact of their new element, hafnium.
References
- Amelin, Y., Lee, D-C., Halliday, A.H., Pidgeon, R.T., 1999. Nature of the Earth’s earliest crust from hafnium isotopes in single detrital zircons. Nature 399, 252-255.
- Bauer, A.M., Reimink, J.R., Chacko, T., Foley, B.J., Shirey, S.B., Pearson, D.G., 2020. Hafnium isotopes in zircons document the gradual onset of mobile-lid tectonics. Geochem. Persp. Lett. 14, 1-6.
- Borg, L.E., Carlson, R.W., 2023. The evolving chronology of Moon formation. Ann. Rev. Earth Planet. Sci. 51, 25-52.
- Correira Neves, J.M., Lopes Nunes, J.E., 1974. High hafnium members of the zircon-hafnon series from the granite pegmatites of Zambezia, Mozambique. Contrib. Mineral. Petrol. 48, 73-80
- Coster, D., Hevesy, G., 1923. On the missing element of atomic number 72. Nature 111, 79.
- Goldschmidt, V.M., Thomassen, L., 1923. Das Vorkommen des Elements No. 72 (Hafnium) im Malakon und Alvit. Norsk geol. tidsskr. 7, 61-68.
- Halliday, A.N., 2000, Hf-W chronometry and inner Solar System accretion rates. Space Sci. Rev. 92, 355-370.
- Hisamatsu, Y., Egashira, K., Maeno, Y., 2022: Ogawa’s nipponium and its re‑assignment to rhenium. Found. Chem. 24, 15-57.
- Marshall, J.L, Marshall, V.R., 2016. Rediscovery of the elements. The rare Earths – the last member. Hexagon, Spring 2016, 4-9.
- Kruijer, T.S, Sprung, P., Kleine, T., Leya, I. Burkhardt, C., Wieler, R., 2012. Hf–W chronometry of core formation in planetesimals inferred from weakly irradiated iron meteorites. Geochim. Cosmochim. Acta 99, 287-304.
- Kruijer, T.S, Kleine, T., Fischer-Gődde, M., Sprung, P. 2015 Lunar tungsten isotopic evidence for the late veneer.Nature 520, 534.
- Kruijer, T.S, Archer, G.J., Kleine, T., 2021. No 182W evidence for early Moon formation. Nature 141, 714-715.
- Kruijer, T.S, Kleine, T., 2017. Tungsten isotopes and the origin of the Moon. Earth Planet. Sci. Lett. 475, 15-24
- Lee, D-C., Halliday, A.N., 1996. Hf-W isotopic evidence for rapid accretion and differentiation in the early solar system. Science 274, 1876-1879.
- Mason, B., 1992. Victor Moritz Goldscmidt: Father of Modern Geochemistry. Geochem. Soc. Spec. Publ. 4, Chapter 5. Intermezzo: The Search for Element 72.
- Moseley, H.J.G., 1913. The high-frequency spectra of the elements (1), Phil. Mag. 26, 1025-1034.
- Moseley, H.J.G., 1914. The high-frequency spectra of the elements (2), Phil. Mag. 27, 703–713.
- Mundl, A., Touboul, M., Jackson, M.G., Day, J.M.D., Kurz, M.D., Lekic, V., Helz, R.T., Walker, R.J., 2017. Tungsten-182 heterogeneity in modern ocean island basalts. Science 356, 66-69.
- Mundl-Petermeier, A., Walker, R.J., Fischer, R.A., Lekic, V., Jackson, M.G., Kurz, M.D., 2020. Anomalous 182W in high 3He/4He ocean island basalts: Fingerprints of Earth’s core? Geochim. Cosmochim. Acta 271, 194-211.
- Noddack, W., Tacke, I., 1925. Die Ekamangane. Naturwissenschaften 13, 567-574.
- Rutherford, E., 1915. Henry Gwyn Jeffreys Moseley. Nature 96, 33-34.
- Shannon, R.D., Prewitt, C.T., 1970. Revised values of effective Ionic radii. Acta Cryst. B. 26, 1046-1048.
- Shannon, R.D., 1976. Revised effective ionic radii and systematic studies of interatomie distances in halides and chaleogenides. Acta Cryst. A. 32, 751-767.
- Speer, J.A., Cooper, B.J., 1982. Crystal structure of synthetic hafnon, HfSiO4, comparison with zircon and the actinide orthosilicates. Am. Mineral. 67, 804-808.
- Touboul, M., Sprung, P., Aciego, S.M. Bourdon, B., Kleine, T., 2014, Hf–W chronology of the eucrite parent body. Geochim. Cosmochim. Acta 156, 106-121.